Moisture, warmth, and aeration; soil texture; soil fitness; soil organisms; its tillage, drainage, and irrigation; all these are quite as important factors in the makeup and maintenance of the fertility of the soil as are manures, fertilizers, and soil amendments.
—J.L. Hills, C.H. Jones and C. Cutler, 1908
The physical condition of a soil has a lot to do with its ability to produce crops, mostly because it anchors their roots. A very fundamental aspect of soil is its ability to hold water between particles and act like a sponge in the landscape. This phenomenon, capillarity (or capillary action), helps store precipitation, thereby making it available to plants and other organisms or transmitting it slowly into groundwater or streams. Also, water in soil allows for the very slow but steady dissolving of soil minerals, which are absorbed by plants and cycled back onto the soil as organic matter. Over the course of many years these small amounts of minerals build up as a pool of stored organic nutrients available for agricultural production.
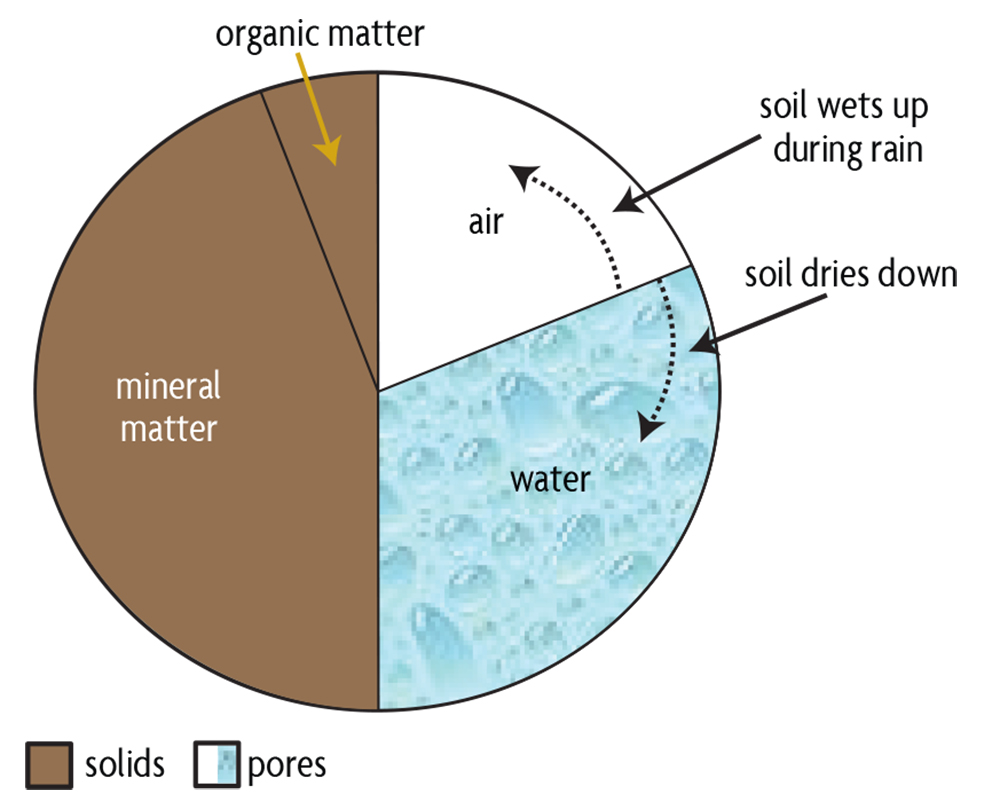
A degraded soil usually has reduced water infiltration and percolation (drainage into the deeper soil), aeration and root growth. These conditions lessen the ability of the soil to supply nutrients, render harmless many hazardous compounds (such as pesticides), and maintain a wide diversity of soil organisms. Small changes in a soil’s physical conditions can have a large impact on these essential processes. Creating a good physical environment, which is a critical part of building and maintaining healthy soils, requires attention and care.
Let’s first consider the physical nature of a typical mineral soil. It usually contains about 50% solid particles on a volume basis (Figure 5.1), with the spaces in between, pores, accounting for the remaining volume. Most solid particles are minerals, and organic matter is a small, but a very important, component of the soil. The soil’s mineral particles are a mixture of variously sized minerals that define its texture. A soil’s textural class, such as a clay, clay loam, loam, sandy loam or sand, is perhaps its most fundamental inherent characteristic, as it affects many of the important physical, biological and chemical processes in a soil. Soil texture changes little over time, no matter how the soil is managed.
Texture, A Basic Soil Property
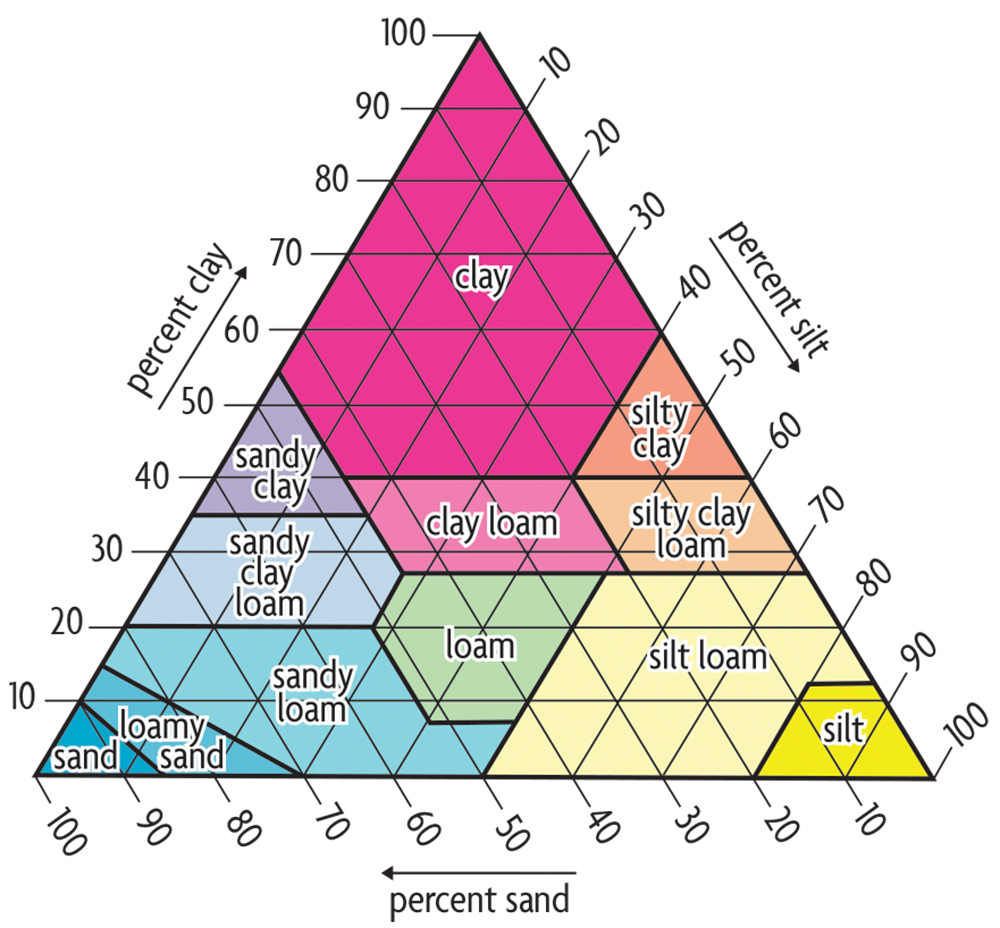
The textural class of a soil (Figure 5.2) indicates the coarseness or fineness of a soil’s particles. It is defined by the relative amounts of sand (.05–2 millimeters particle size), silt (.002–.05 millimeters) and clay (less than 0.002 millimeters). Particles that are larger than 2 millimeters are rock fragments (pebbles, cobbles, stones and boulders), which are not considered in the textural class because they are relatively inert. Soil particles are the building blocks of the soil skeleton. But the spaces between the particles and between aggregates are just as important as the particles themselves because that’s where most physical and biological processes happen. The quantity of variously sized pores—large, medium, small and very small—govern the important processes of water and air movement. Also, soil organisms live and function in pores, which moreover is where plant roots grow. Most pores in clay are small (generally less than 0.002 millimeters), whereas most pores in sandy soil are large (but generally still smaller than 2 millimeters). The pore sizes are affected not only by the relative amounts of sand, silt and clay in a soil, but also by the amount of aggregation. On the one extreme, we see that beach sands have large particles (in relative terms, at least—they’re visible) and no aggregation due to a lack of organic matter or clay to help bind the sand grains. A good loam or clay soil, on the other hand, has smaller particles, but they tend to be aggregated into crumbs that have larger pores between them and small pores within. Although soil texture doesn’t change over time, the total amount of pore space and the relative amount of variously sized pores are strongly affected by management practices. Aggregation and structure may be destroyed or improved depending on, for example, how much tillage occurs, whether good rotations are followed, or if cover crops are used.
Water and Aeration
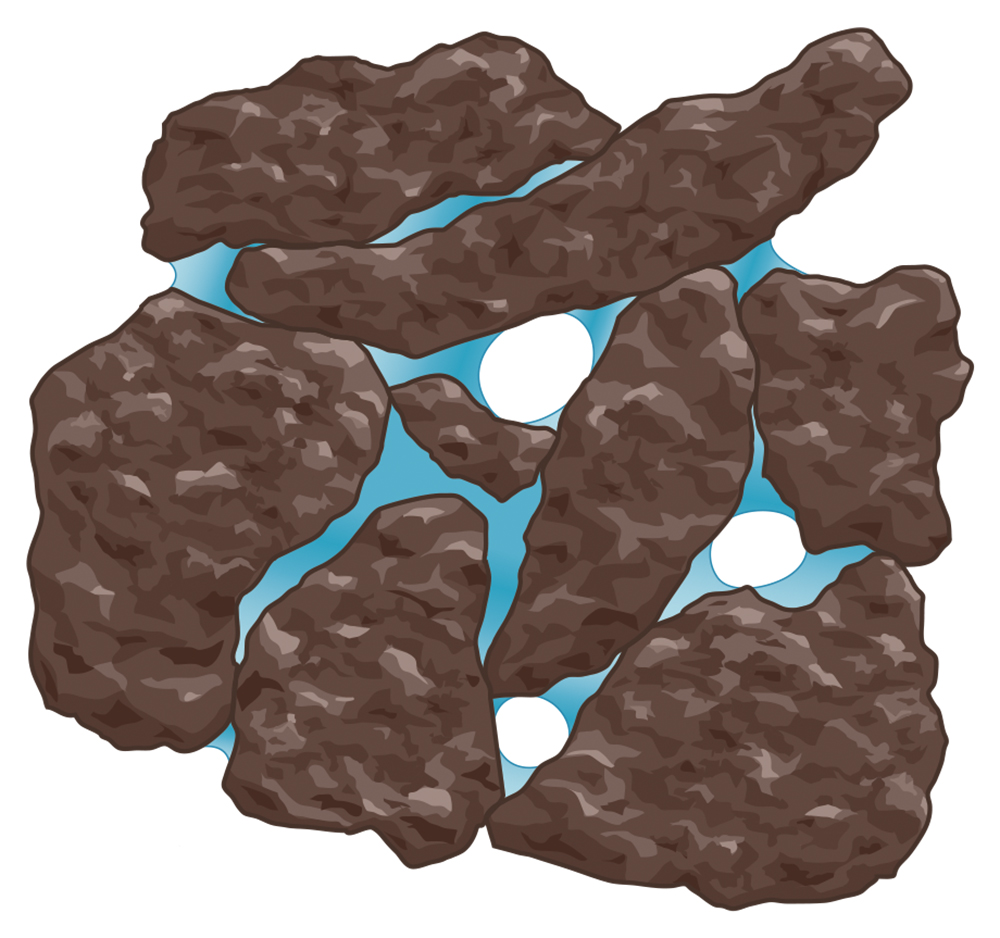
Soil pore spaces are generally filled with water, air, and biota. Their relative amounts change as the soil wets and dries (figures 5.1, 5.3). On the wet extreme, when all pores are filled with water, the soil is water saturated and the exchange of gases between the soil and atmosphere is very slow. During these conditions, carbon dioxide produced by respiring roots and soil organisms can’t escape from the soil and atmospheric oxygen can’t enter, leading to undesirable anaerobic (no oxygen) conditions. On the other extreme, a soil with little water may have good gas exchange but may be unable to supply sufficient water to plants and soil organisms. Water in soil is mostly affected by two opposing forces that basically perform a tug of war: Gravity pulls water down and makes it flow to deeper layers, but capillarity holds water in a soil pore because it is attracted to solid surfaces (adhesion) and has a strong affinity for other water molecules (cohesion). The latter are the same forces that keep water drops adhering to glass surfaces, and their effect is strongest in small pores (Figure 5.3) because of closer contact with solids.
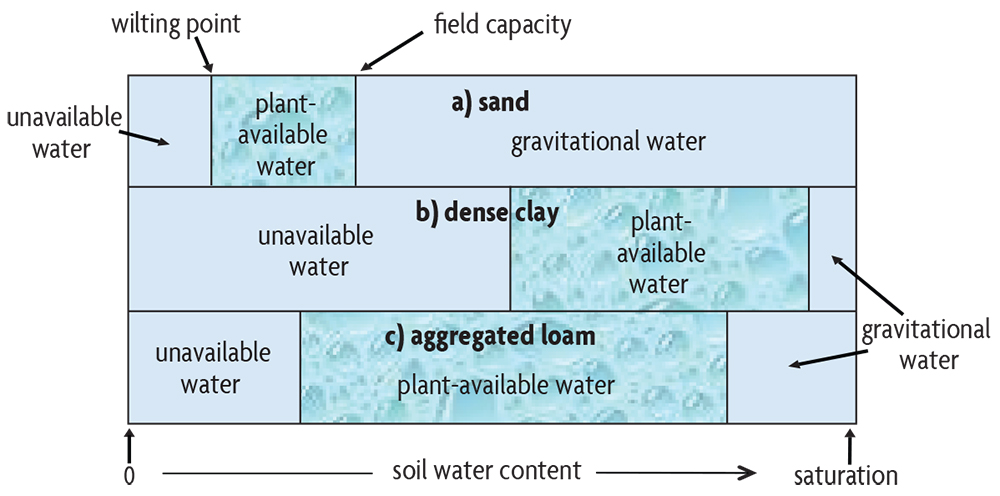
Soils are thus a lot like sponges in the way they hold and release water (Figure 5.4). When a sponge is fully saturated, it quickly loses water by gravity but will stop dripping after about 30 seconds. The largest pores drain rapidly because they are unable to retain water against the force of gravity. But when it stops dripping, the sponge still contains a lot of water in the smaller pores, which hold it more tightly. This water would, of course, come out if you squeezed the sponge. Its condition following free drainage is akin to a soil reaching its so-called field capacity water content, which in the field occurs after about two days of free drainage following saturation by a lot of rain or irrigation. If a soil contains mainly large pores, like a coarse sand, most pores empty out quickly and the soil loses a lot of water through quick gravitational drainage. Therefore the soil’s field capacity water content is low.
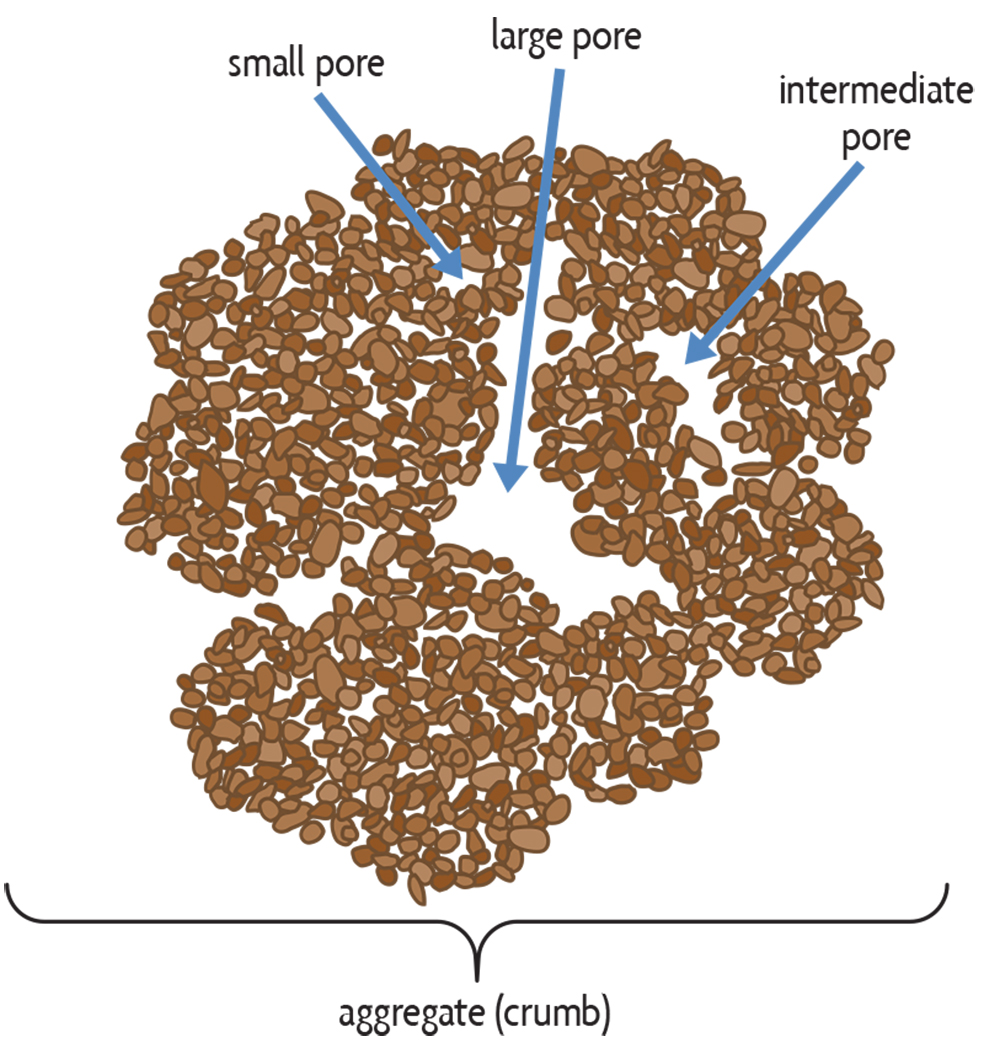
This drainage is good because the pores are now open for air exchange. On the other hand, little water remains for plants to use, resulting in more frequent periods of drought stress. Therefore, coarse sandy soils have very small amounts of water available to plants before they reach their wilting point (Figure 5.4a). Also, the rapidly draining sands more readily lose dissolved chemicals in the percolating water (pesticides, nitrate, etc.), but this is much less of a problem with fine loams and clays. A dense, fine-textured soil, such as a compacted clay loam, has mainly small pores that tightly retain water and don’t release it. It therefore has a high field capacity water content, and the more common anaerobic conditions resulting from extended saturated conditions cause other problems, like gaseous nitrogen losses through denitrification, as we will discuss in Chapter 19.
The ideal soil is somewhere between the two extremes, and its behavior is typical of that exhibited by a well-aggregated loam soil (figures 5.4c, 5.5). Such a soil has a sufficient amount of large pore spaces between the aggregates to provide adequate drainage and aeration during wet periods, but also has enough small pores and waterholding capacity to provide water to plants and soil organisms between rainfall or irrigation events. Besides retaining and releasing water at near optimum quantities, such soils also allow for good water infiltration, thereby increasing plant water availability and reducing runoff and erosion. This ideal soil condition is therefore characterized by medium texture and crumb-like aggregates, which are common in good topsoil.
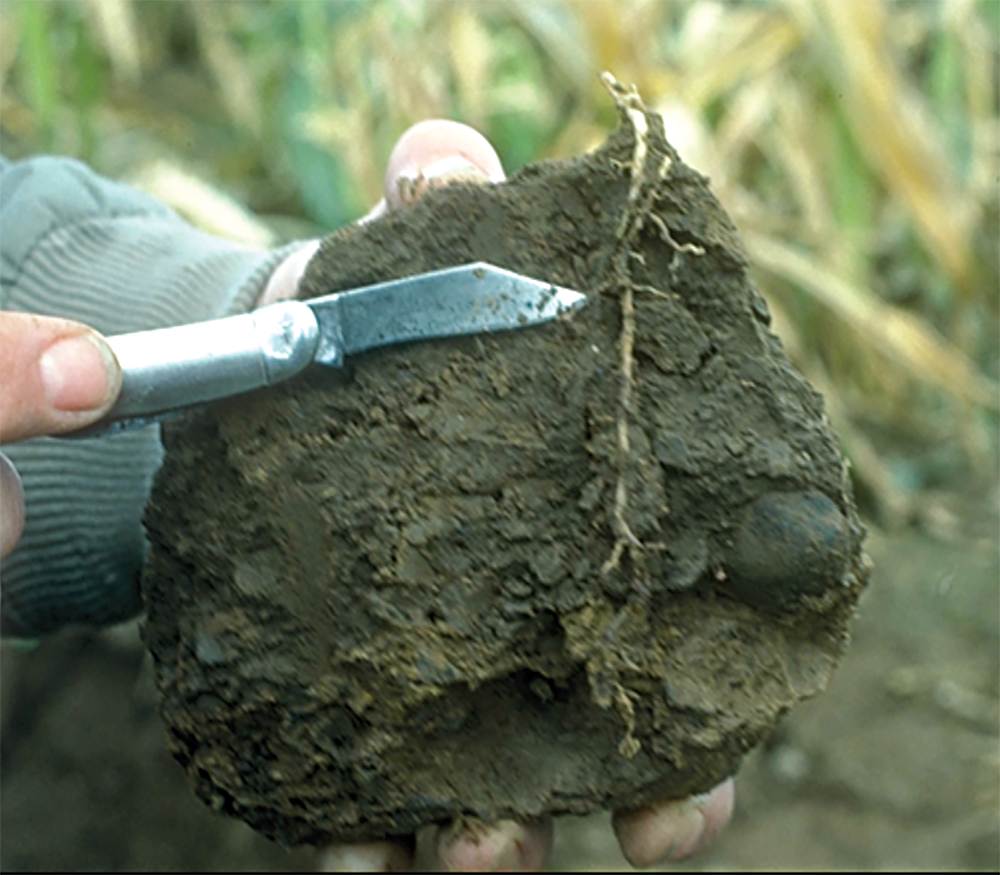
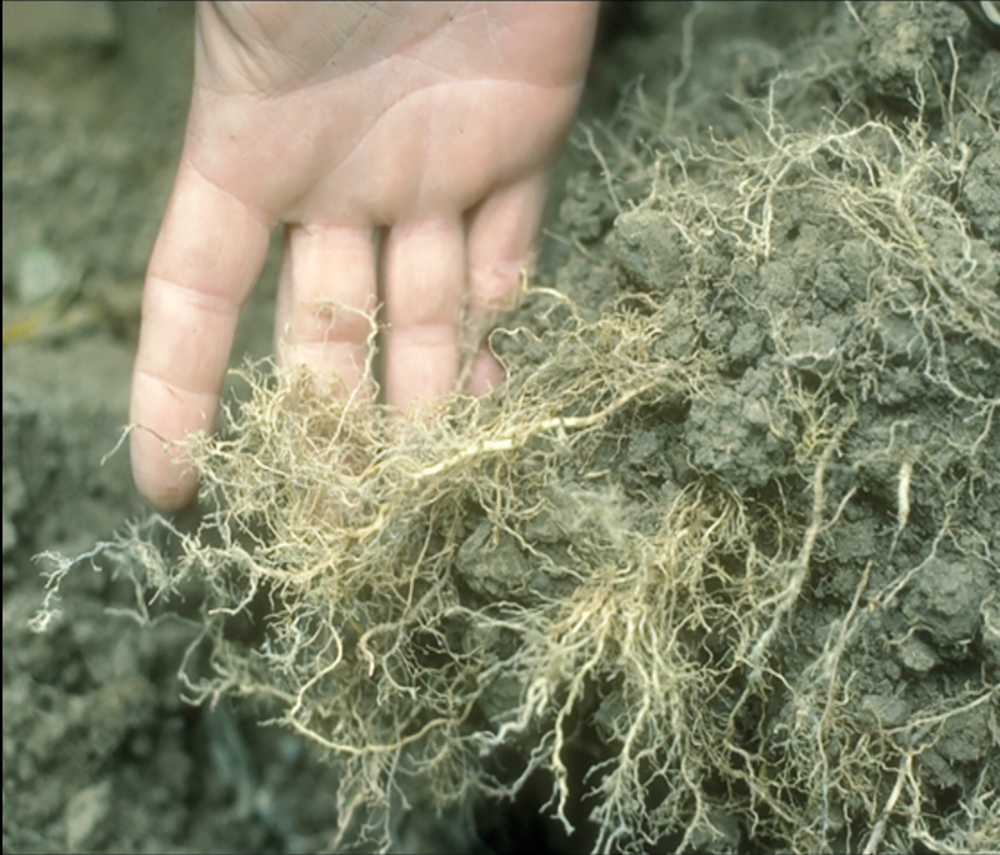
Available Water and Rooting
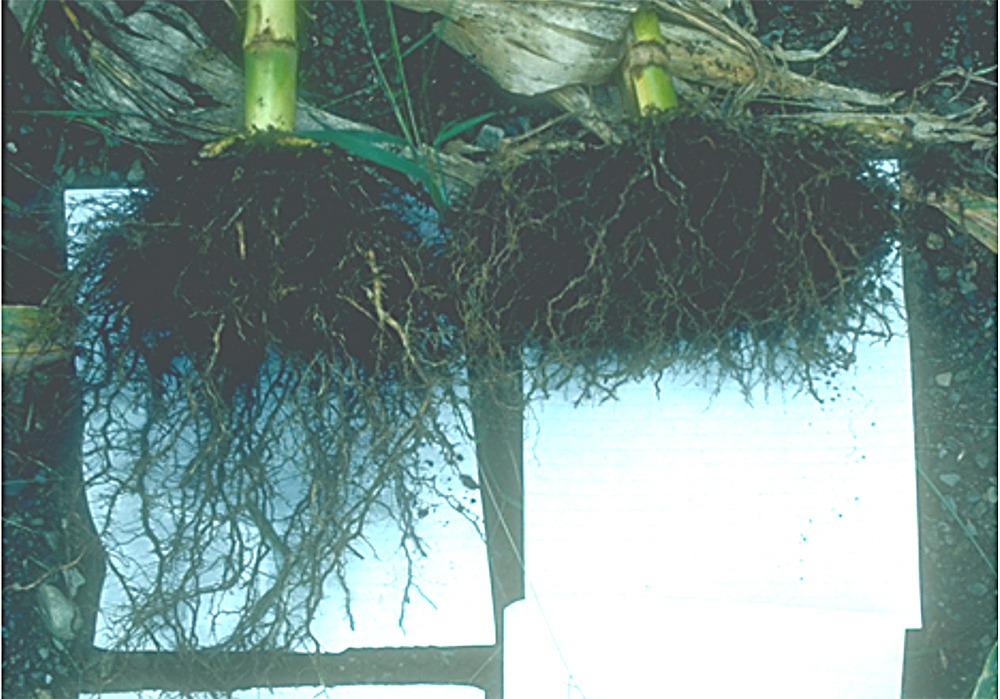
There is an additional dimension to plant-available water capacity of soils: The water and nutrients not only need to be stored and available in the soil pores, but roots also need to be able to access them. This may be a problem if the soil is compacted. Consider the soil from the compacted surface horizon in Figure 5.6 (left), which was penetrated only by a single corn root with few fine lateral rootlets. The soil volume holds sufficient water, which in principle would be available to the corn plant, but the roots are unable to penetrate most of the hard soil volume. The corn plant, therefore, could not obtain the moisture and nutrients it needed. Conversely, the corn roots on the right (Figure 5.6) are able to fully explore the soil volume with many roots, fine laterals, root hairs and mycorrhizal fungi (not shown) allowing for better water and nutrient uptake.
Similarly, the depth of rooting can be limited by compaction. Figure 5.7 shows, on the right, corn roots from moldboard-plowed soil with a severe plow pan (a hard layer right below the depth of tillage). The roots cannot penetrate into the subsoil and are therefore limited to water and nutrients in the plow layer near the surface. The corn on the left is grown in soil that was subsoiled, and the roots are able to reach about twice the depth. Subsoiling opens up more soil for root growth and, therefore, more usable water and nutrients. Thus, plant water availability is a result of both the soil’s water retention capacity (related to texture, aggregation and organic matter) and potential rooting volume, which is strongly influenced by compaction.
Crop Water Needs
Different crops need different amounts of water, supplied by precipitation or by irrigation. For example, crops like alfalfa require a lot of water for maximum yields and the plant’s long taproot helps it access water deep in the soil. On the other hand, vineyards and crops such as wheat need much smaller amounts of water. And many crops such as corn and potatoes are in between in their water needs. This may influence farmers’ choice of crops to grow as some regions of the United States and other parts of the world are projected to become drier and warmer as the climate changes and water for irrigation becomes harder to obtain.
Infiltration Versus Runoff
An important function of soil is to absorb water at the land surface and either store it for use by plants or slowly release it to groundwater through gravitational flow (Figure 5.8). When rainfall hits the ground, most water will infiltrate the soil, but under certain conditions it may run off the surface or stand in ruts or depressions before infiltrating or evaporating. The maximum amount of rainwater that can enter a soil in a given time, called infiltration capacity, is influenced by the soil type (large pores result in higher capacity), structure and moisture content at the start of the rain.
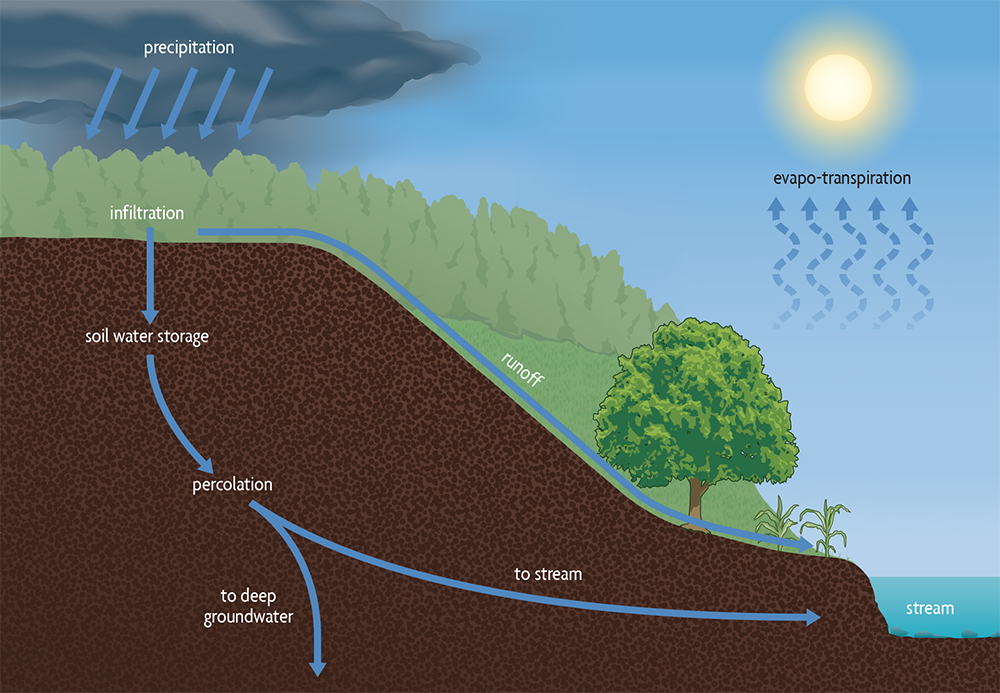
If rain is very gentle, the infiltration capacity is generally not exceeded and all precipitation enters the soil. Even in an intense storm, water initially enters a soil readily as it is literally sucked into the dry ground. But as the soil wets up during a continuing intense storm, water entry into the soil is reduced and a portion of rainfall may begin to run downhill over the surface to a nearby stream or wetland. The ability of a soil to maintain high infiltration rates, even when saturated, is related to the sizes of its pores. Since sandy and gravelly soils have more large pores, they maintain better infiltration during a storm than fine loams and clays. But soil aggregation is also important in governing the number of pores and their sizes: When finer-textured soils have strong aggregates due to good management, they can also maintain high infiltration rates. But this is not the case when those aggregates fail and the soil becomes compacted.
Runoff is produced when rainfall exceeds a soil’s infiltration capacity. Rainfall or snowmelt on frozen ground generally poses even greater runoff concerns, as pores are blocked with ice. Runoff happens more readily with poorly managed soils because they lack strong aggregates that hold together against the force of raindrops and moving water and, therefore, have few large pores open to the surface to quickly conduct water downward. Such runoff can initiate erosion, with losses of nutrients and agrochemicals as well as sediment.
Soil Water and Aggregation
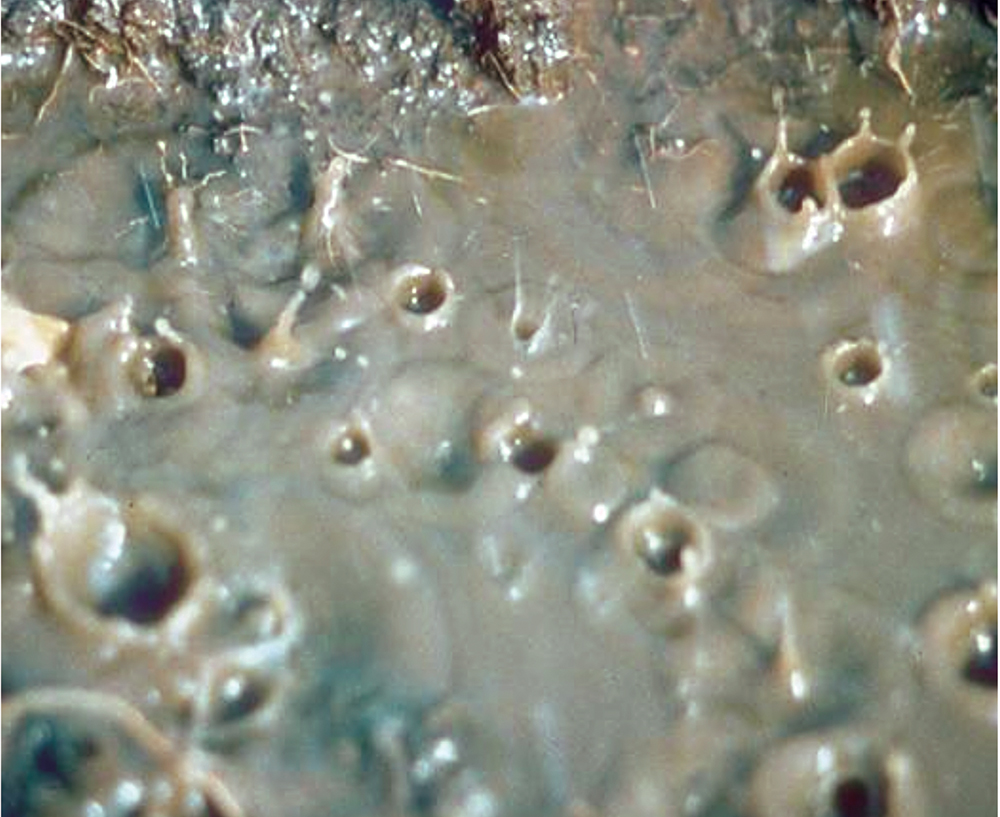
Processes like erosion, soil settling and compaction are affected by soil moisture conditions, and in turn affect soil hardness and the stability of aggregates. When soil is saturated and all pores are filled with water, the soil is very soft. (Fungal hyphae and small roots also serve to form and stabilize aggregates deeper in the soil.) Under these saturated conditions, the weaker aggregates may easily fall apart from the impact of raindrops and allow the scouring force of water moving over the surface to carry soil particles away (Figure 5.9). Supersaturated soil has no internal strength, and the positive water pressure in fact pushes particles apart (Figure 5.10, left). This makes soil very susceptible to erosion by water flowing over the surface or allows it to be pulled down by gravity as land (mud) slides.
As soil dries and becomes moist instead of wet, the pore water remaining in contact with solid surfaces becomes curved and pulls particles together, which makes the soil stronger and harder (Figure 5.10, middle). But when soils low in organic matter and aggregation, especially sands, are very dry, the bonding between particles decreases greatly because there is no pore water left to hold the particles together. The soil then becomes loose and the shear force of wind may cause particles to become airborne and cause wind erosion (Figure 5.10, right).
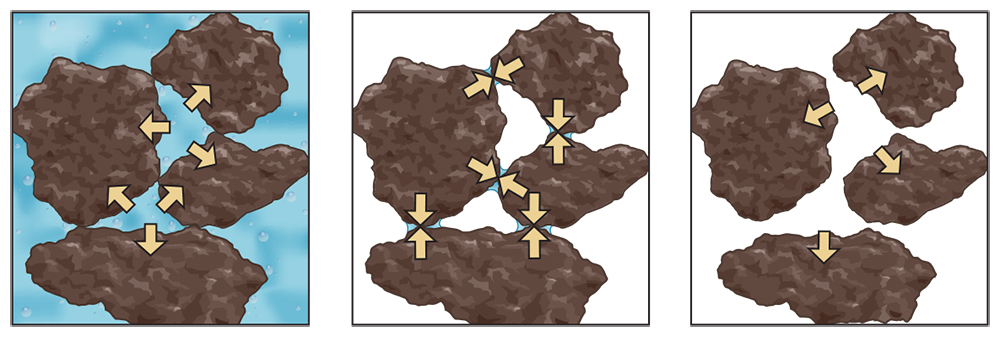
Strong aggregation is especially important during these moisture extremes, as it provides another source of cohesion that keeps the soil together. Good aggregation, or structure, helps to ensure a high-quality soil and prevents dispersion (Figure 5.11). A well-aggregated soil also results in good soil tilth, implying that it forms a good seedbed after soil preparation. Aggregation in the surface soil is enhanced by mulching or by leaving residue on the surface, and also by limiting or eliminating tillage. A continuous supply of organic materials, roots of living plants and mycorrhizal fungi hyphae are also needed to maintain good soil aggregation.
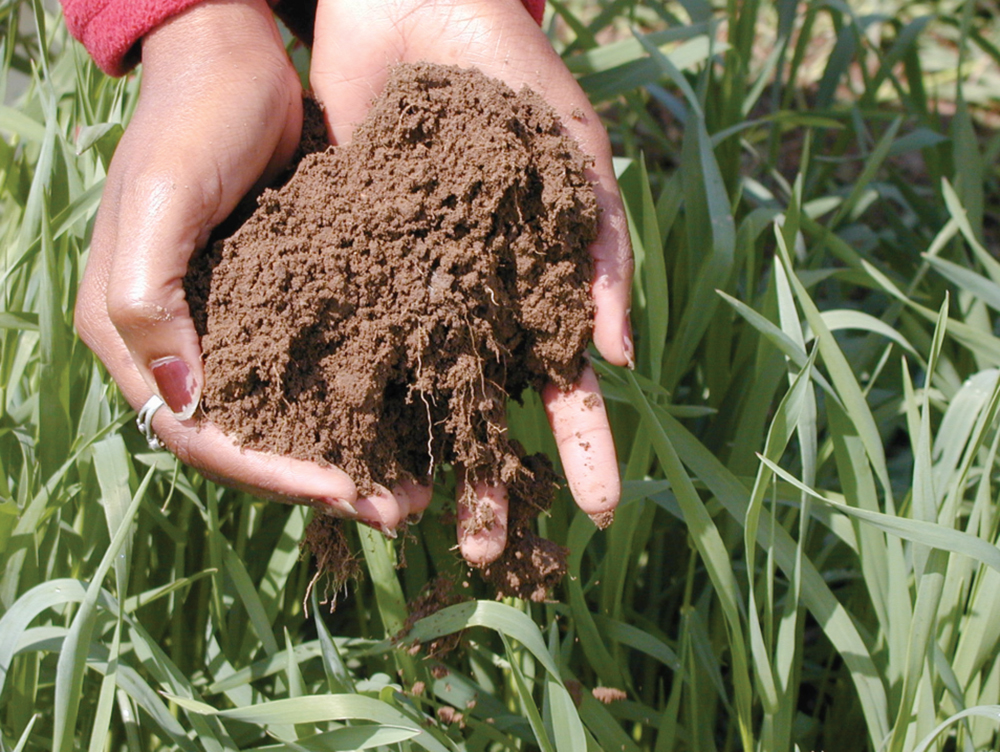
Surface residues and cover crops protect the soil from wind and raindrops and moderate the temperature and moisture extremes at the soil surface. Conversely, an unprotected soil may experience very high temperatures at the surface and become extremely dry. Worms and insects will then move deeper into the bare soil, which results in a surface zone that contains few active organisms. Many bacteria and fungi that live in thin films of water may die or become inactive, slowing the natural process of organic matter cycling. Large and small organisms promote aggregation in a soil that is protected by a surface layer of crop residue cover, mulch or sod and has continuous supplies of organic matter to maintain a healthy food chain. An absence of both erosion and compaction processes also helps maintain good surface aggregation.
The soil’s chemistry also plays a role in aggregate formation and stability, especially in dry climates. Soils that have high sodium content (see Chapter 6 and Chapter 20) pose particular challenges.
What Comes From the Sky: The Lifeblood of Ecosystems
We need to take a short diversion from our focus on soils and briefly discuss climate. Various characteristics of precipitation affect the potential for crop production and the losses of water, sediment and contaminants to the environment. These include the annual amount of precipitation (for example, an arid versus humid climate); the seasonal distribution and relation to the growing season (wet seasons and dry seasons; can rainfall supply the crops or is irrigation routinely needed?); and the intensity, duration and frequency of rain (regular gentle showers are better than infrequent intense storms that may cause runoff and erosion).
Precipitation patterns are hardly ever ideal, and most agricultural systems have to deal with shortages of water at some time during the growing season, which remains the most significant yield-limiting factor worldwide. Water excess can also be a big problem, especially in humid regions or monsoonal tropics. In that case the main problem is not the excess water itself but the lack of air exchange and oxygen. Many management practices focus on limiting the effects of these climatic deficiencies. Subsurface drainage and raised beds remove excess water and facilitate aeration; irrigation overcomes inadequate rainfall; aquatic crops like rice allow for grain production in poorly drained soil; and so forth. (See Chapter 17 for a discussion of irrigation and drainage.) So, climate affects how soils function and the processes occurring in soils. What is perhaps less understood is that good soil management and healthy soils are important to reducing susceptibility to climatic vagaries and making soils and crops more resilient to weather extremes. The Great Plains area of the United States learned this during the Dust Bowl era of the 1930s, when a decade of drought and unsustainable soil management practices resulted in excessive wind and water erosion, crop failures, the collapse of the agricultural industry, and massive human migrations out of the region. That devastating experience gave birth to the soil conservation movement, which has achieved much; but most soils, even in the United States, are still in need of protection from erosion, which requires good soil management practices.
Climate Risk and Resilience
The concept of risk integrates the cost of an adverse event with the chance of it occurring. With increasing frequency of weather extremes, the risk of costly or catastrophic events affecting farms and communities goes up. Their vulnerability is characterized by three aspects:
- Exposure: weather-related challenges you are likely to face
- Sensitivity: how and to what degree those events threaten your operation
- Adaptive capacity: how well you can minimize weather-related damage and take advantage of new opportunities
Generally, exposure to extreme weather events is a given, although farmers can help reduce overall greenhouse gas emissions through better cropping systems and nutrient management. Sensitivity to adverse weather events can be addressed through many of the practices we discuss in this book, as well as through other strategies, such as building soil health and thereby enhancing crop vigor while reducing runoff and crop drought stress; diversifying crop and livestock systems to spread risk from an extreme event; incorporating climate risk management into farm planning; building skills and experience with farm staff; installing physical infrastructure like irrigation or drainage; building social networks that allow you to respond better to adverse events; and managing finances and insurance to absorb setbacks.
By building an overall resilient farm operation you reduce potential damages and allow for faster recovery from weather-related disruptions. Still, after-the-fact adaptations still need to be anticipated, like growing an alternative crop when your initial crop was lost and using a weather-adaptive nitrogen management tool.
Adapted from Lengnick (2015)
Chapter 5 Sources
Brady, N.C. and R.R. Weil. 2008. The Nature and Properties of Soils, 14th ed. Prentice Hall: Upper Saddle River, NJ.
Hill, R.L. 1990. Long-term conventional and no-tillage effects on selected soil physical properties. Soil Science Society of America Journal 54: 161–166.
Karunatilake, U. and H.M. van Es. 2002. Temporal and spatial changes in soil structure from tillage and rainfall after alfalfa-corn conversion in a clay loam soil. Soil and Tillage Research 67: 135–146.
Kay, B.D. 1990. Rates of change of soil structure under cropping systems. Advances in Soil Science 12: 1–52.
Lengnick, L. 2015. Resilient Agriculture: Cultivating Food Systems for a Changing Climate, New Society Gabriola Island, Canada. Available in summary at https://www.sare.org/resources/cultivating-climate-resilience-on-farms-and-ranches/.
Nunes, M., H. van Es, E. Pauletto, J.E. Denardin and L.E. Suzuki. 2018. Dynamic changes in compressive properties and crop response after chisel tillage in a highly weathered soil. Soil & Tillage Res. 186: 183–190.
Nunes, M., R.R. Schindelbeck, H.M. van Es, A. Ristow and M. Ryan. 2018. Soil Health and Maize Yield Analysis Detects Long-Term Tillage and Cropping Effects. Geoderma 328: 30–43.
Shepard, G., C. Ross, L. Basher and S. Suggar. Visual Soil Assessment, vol. 2: Soil Management Guidelines for Cropping and Pastoral Grazing on Flat to Rolling Country. Horizons.mw and Landcare Research: Palmerston North, New Zealand.Whitman, H., ed. 2007. Healthy Soils for Sustainable Vegetable Farms: Ute Guide. Land and Water Australia, AUSVEG, Ltd.: Clayton North, Victoria.