As a geological and biological matrix, healthy, living soil is protected and buffered against dynamic changes or degradation. However, physical or chemical disruption of the soil environment destroys the healthy function between minerals, water, gases, roots and animals. Landslides, floods and storms are naturally occurring disruptions to soils. Many conventional practices used in growing crops are damaging to soil health—in the short term and long term. External inputs or additional disturbance only mask the damage temporarily and are economically and ecologically costly. This chapter describes the negative effects on soil health caused by tillage, climate change, synthetic fertilizer applications and pesticides. Chapter 5 covers practices that can build and protect soils that are healthy and alive.
Tillage
Tillage is destructive to the physical structure of soil and the living organisms within it. The primary reasons for tillage have been to destroy weeds and to achieve consistent planting conditions, but much more is destroyed in the process. The physical disturbance of tillage breaks up soil structure and destroys aggregates and soil pores. (Inversion tillage is the most destructive; plows, disks, shanks and vertical tillage tools are increasingly less destructive.) Soil structure is critical to water infiltration, root growth, and nutrient and gas exchange. Existing channels from dead roots and earthworm or animal tunnels are lost with tillage. This impacts water infiltration and storage, as well as the habitat value for animals that use the tunnels.
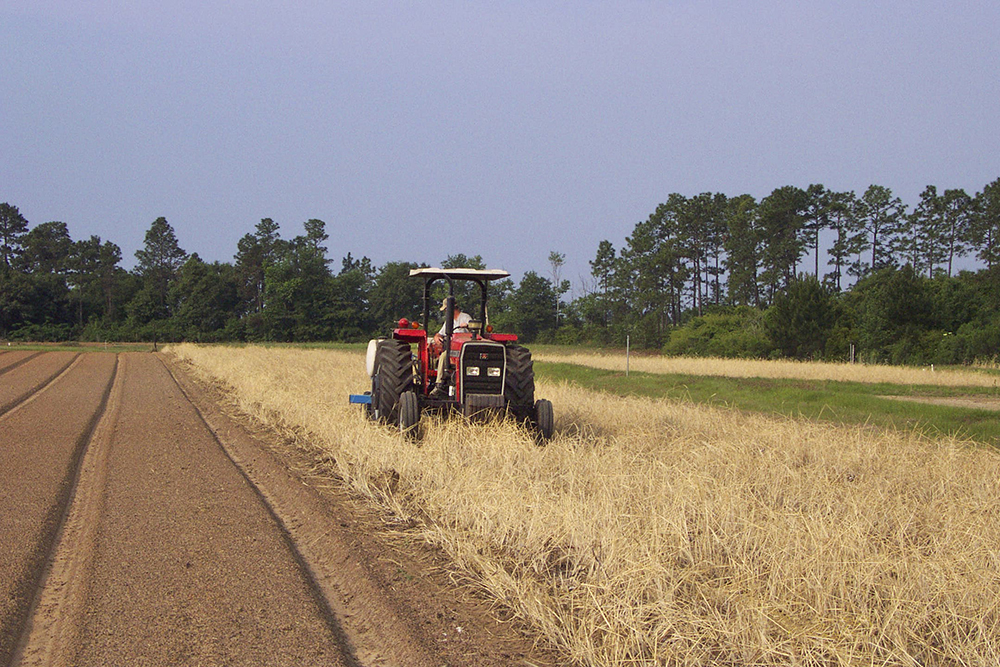
When surface aggregates are destroyed, the fine particles form a crust that inhibits infiltration and impedes seedling emergence. Tillage churns soil and dramatically increases the exposure of soil surfaces to the atmosphere. This exposes carbon, previously held inside aggregates, to microbes in an oxygen-rich environment. Microbes quickly eat this carbon, and much is lost as carbon dioxide. The loss of soil cover and the exposure of dark soil surfaces to heating by the sun increases soil temperatures. Higher soil temperatures can be detrimental to many soil macrofauna, mesofauna, and microfauna and result in the loss of soil water needed by some animals to move around within the soil.
Tillage results in shifts in detrital food webs: undisturbed soils support extensive fungal networks, and fungi form the basis for detrital food webs in untilled soils, but plowed soils tend to shift toward bacterial decomposers at the base of the detrital food web.
Climate Change
The effect of climate change on soil health and soil organisms depends on a variety of factors, such as geographic region (variable and associated climate change projections), soil type and land management practices. Most areas in the United States will experience an increase in extreme weather events, including droughts, flooding, and intense storms that can affect soil systems. Many areas, including much of the eastern half of the United States, will experience an increase in extreme rainfall events, which can lead to soil erosion. In the northeastern United States, precipitation intensity and amounts are expected to increase more than most other regions in the United States. In other areas, such as California and the Great Basin, drought will become more common and increasingly unpredictable. Some areas will have increased frequency and duration of flooding. Flooding can scour and wash away soil, and where water sits for days or more, the inundated soils have poor oxygen, which leads to plant and animal death. Increased drought and heat reduce plants’ ability to persist, which could leave soils bare and exposed to erosion and result in a cascade of loss of soil animals that depend on plants for food and habitat. The general instability or unpredictability of seasonal temperatures will affect the timing of flowering and life cycles of insects and microbes. Sea level rise will increase the risk of flooding in coastal areas, where inundation with saltwater will be a problem for soil health and farmland productivity.
While climate change can have adverse effects on soil health, especially in systems where soil health is already poor or degraded, soils also have an important role to play in mitigating climate change. Healthy soils sequester more carbon than degraded soils, which means that improving soil health can turn farmland into a climate sink (land that stores more carbon than it releases). Thus, farmers have the potential to lead the fight against climate change—by implementing practices that prioritize soil health, farms become a key part in mitigating the climate crisis. All of the practices described in Chapter 5: Farming Practices that Support Soil Health will improve soil health and improve the carbon sequestration services of soil on your farm.
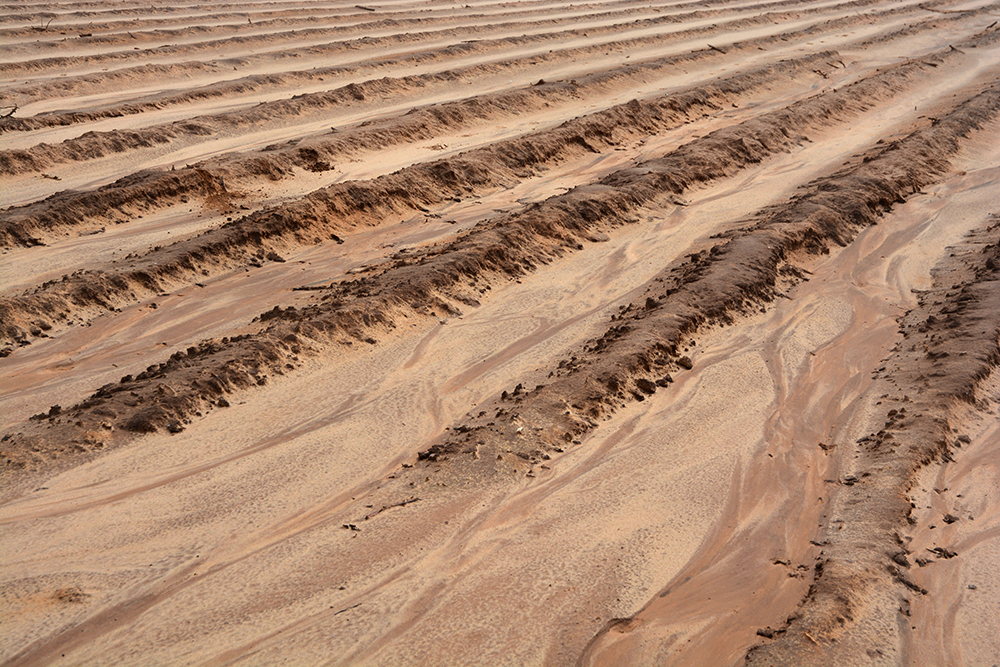
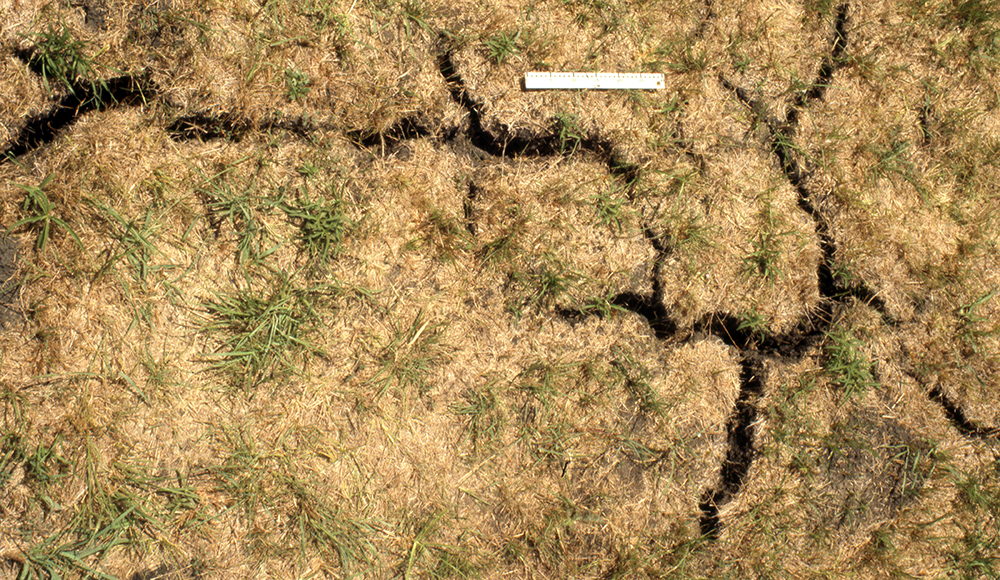
Synthetic Fertilizers
Inorganic, synthetic fertilizer application creates spikes in the abundance of available nitrogen, phosphorus and potassium, disrupting the processes of decomposition and nutrient cycling. The response of microbes to the nutrients affects available soil carbon and the diversity and abundance of soil animals. Some groups tend to increase in abundance following nitrogen fertilizer application, such as microbes and animals associated with plant roots and organic matter, while microarthropods decrease. However, long term use of nitrogen fertilizers—particularly ammonium-based N fertilizers—can accelerate soil acidification, which can in turn affect soil microbial communities. Synthetic fertilizers create an imbalance in the natural functioning of soil. USDA soil scientist Rick Haney compares synthetic fertilizer use to a diet of only vitamins—without fiber (organic matter), the system can’t be healthy in the long term. Sustained nutrient cycling and availability depends upon interconnected soil organisms and communities.
Pesticides
Pesticides—which include insecticides, fungicides, herbicides and soil fumigants—are used to control insects, diseases, weeds and other pests that can reduce the growth or productivity of agricultural crops. More than one billion pounds of pesticide active ingredients are used annually in the United States, with more than 90% of that usage in agricultural settings.
Impacts of Pesticides on Soil Invertebrates
A variety of pesticides can disrupt soil microbial and invertebrate communities, as well as the soil functions and processes these organisms mediate. Effects of pesticides in soils can range from lethal, including immediate death or shortened life span, to sublethal, such as diminished health, behavior, reproduction, and growth. Pesticides can also affect soil fauna indirectly by disturbing the soil food web and community dynamics (e.g., by reducing prey for natural enemies).
Pesticide residues in soils of crop fields and field margins are a possible route of exposure for the nearly 70% of bee species that nest in soil. Highly mobile systemic insecticides and fungicides move easily from treated fields into the soils and plants of adjacent habitats, including from dust off during the planting of treated seeds as well as movement through soils. (See "Neonicotinoid Insecticides" on page 28 for more information on neonicotinoids, a class of systemic insecticides.) Neonicotinoid residues in the soils of agricultural field margins have been associated with lower richness in bee species visiting those areas. Fungicide contamination could affect larval nutrition and development and make bees more susceptible to other stressors such as insecticide exposure and pathogen infection. Chronic exposure to pesticide residues—for example, bee larvae exposed to contaminated soils—could have a variety of impacts on development, longevity and reproduction.
Earthworms and potworms, which provide key soil functions and represent the majority of soil faunal biomass in most terrestrial ecosystems, are impacted by pesticides at individual, community, and population levels—some insecticides, fungicides and herbicides can increase these animals’ individual mortality, reduce their growth and reproduction, change individual behavior (such as feeding rate), or decrease the overall community biomass and density. These effects can be mixed and sometimes short-lived, but chronic pesticide exposure in soils is likely to have long-term implications for soil life. Soil communities are likely to exhibit short- and long-term shifts in species composition, favoring soil life that are less susceptible to or that can more readily detoxify pesticides.
A soil organism’s degree of exposure to soil pesticides depends on its lifestyle. Soil-dwelling invertebrates that are highly mobile, such as predatory arthropods, are more likely to be exposed as they move through contaminated soils than soil organisms that are fairly stationary. Invertebrates that live close to the soil surface are also more likely to be exposed to pesticides than those that live deeper below the surface.
In the long term, populations and species may adapt in order to survive in contaminated soils, including increasing expression of certain detoxification enzymes. However, these adaptations may come at an unknown energetic, behavioral or reproductive cost.
It’s important to remember that soil invertebrates and the ecological processes they mediate are not the only animals and processes affected by pesticides applied to the soil. Pesticide residues taken up and stored in the tissues of earthworms and other invertebrates can move up food chains, accumulating at higher doses in the birds and other wildlife that eat these soil-dwelling animals. One of the main legacies of the insecticide DDT was its devastating impacts on birds of prey. Modern insecticides, such as neonicotinoids, appear to be having similar cascading negative impacts on insectivorous birds. Pesticide use also poses risks to pesticide applicators, farmworkers and other communities that are regularly exposed to pesticides. Agricultural workers experience pesticide injury and illness at rates many times higher than the general population, and both acute and chronic exposure to pesticide residues can result in negative health outcomes for farmworkers and their families. Adoption of nonchemical management strategies for insect, disease and weed management benefits both human health and the environment.
Fate of Pesticides in Soil
Depending on the method and timing of application, some or all of the pesticides applied to crops can end up in the soil. Some pesticides are applied directly to the soil as fumigants, granules or pellets spread on the soil surface; soil drenches and injections; or seed coatings. Pesticides sprayed on plant foliage or via aerial applications can also move into soil and groundwater; for example, approximately 65% of high-volume sprays applied by ground equipment to blueberry bushes for mite control were found to pass through the bushes into the soil. Pesticides can also enter the soil from crop residues, leaf fall and root exudates.
Pesticide interactions with soil organic matter and life are complex. Some pesticides bind to soil particles— primarily organic matter—while others may be taken up by plants or leached through the soil profile into water bodies. Adsorption (binding) to soil particles involves a variety of bonding mechanisms and strengths; only some pesticide residues in soil are bioavailable to plants and soil organisms.
Soil microorganisms largely mediate the degradation of pesticides in soil. Bacteria, fungi, algae and other microbes in the soil metabolize or otherwise catalyze the transformation of pesticide active ingredients into less bioactive substances. At the soil surface, light and heat also work to break down pesticides. Persistence of different pesticides in the soil environment depends on many interacting factors, including climate, soil type, type or nature of the pesticide, and whether it was applied once or several times. Degradation is generally much slower in dry soils. Soil amendments like compost and manure can affect the fate of pesticides. Increasing organic matter content may increase the adsorptive capacity of soils, as well as microbial activity and associated decomposition of pesticides. Tillage also interacts with pesticide residues in soil—some pesticides are intentionally incorporated into soil through tillage, and in other cases, tillage that brings pesticide residues to the soil surface may lead to volatilization (evaporation of residues into the atmosphere) or degradation of those residues. Pesticides that volatilize can move far from where they were applied as vapor drift before settling back out of the atmosphere.
The NRCS offers a pesticide risk screening tool, Windows Pesticide Screening Tool (WIN-PST). This tool can help evaluate the potential for a pesticide to leach or run off at a site based on the pesticide’s physicochemical characteristics and the site’s specific conditions, such as soil type and slope. The downloadable WIN-PST database (see Resources) includes a variety of information about the toxicity and environmental fate of currently registered pesticides.
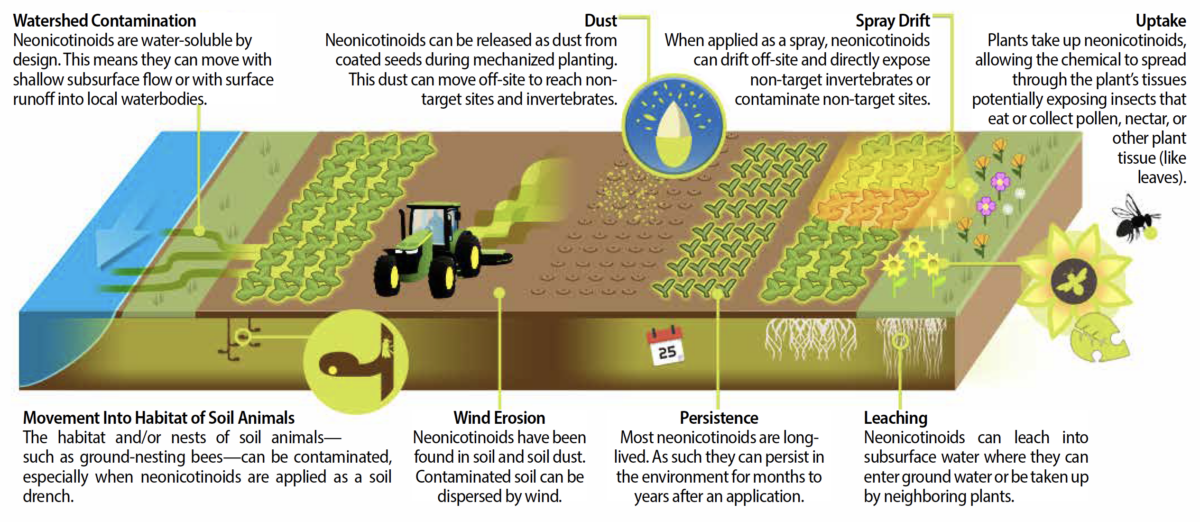
Insecticides
Insecticides, particularly broad-spectrum insecticides with a mode of action affecting many different types of insects, are generally the most toxic pesticides to arthropods in the soil. Broad-spectrum insecticides such as organophosphates, carbamates, avermectins, neonicotinoids and synthetic pyrethroids can reduce the abundance, species richness or biomass of predatory arthropods, isopods, earthworms and potworms in the soil. Insecticides can have complex ripple effects in soil food webs: loss of predators following insecticide applications can result in population spikes of their prey. Sometimes these population spikes become secondary pest outbreaks; pests that were previously suppressed by natural enemies reach a population level at which they cause economic damage to crops.
Systemic insecticides, including neonicotinoids, sulfoximines and anthranilic diamides, are water-soluble pesticides that move easily within plants and can be translocated from the site of application to other parts of the plant. Typically, systemic insecticides are applied to soil and taken up by plant roots, but they can also be applied to foliage or injected directly into woody plants. Because these chemicals dissolve easily in water, they can be quite mobile in soils and prone to leaching and off-site movement. Large-scale use of systemic insecticides in agriculture has raised concern about negative impacts on decomposition, nutrient cycling, soil respiration and invertebrate populations.
Studies of neonicotinoid seed treatments have found mixed impacts on soil microbial activity and community composition, with some studies indicating that effects of seed treatments are short-term and even stimulatory, and others finding adverse effects on soil microbial communities and their function (e.g., lower respiration rate or metabolic activity, and shifts in community composition or diversity).
Neonicotinoid Insecticides
Neonicotinoids are the most widely used class of insecticides worldwide, representing more than 25% of total global insecticide sales. Neonicotinoids pose a particular risk to soil invertebrate communities due to their high toxicity to most insects, systemic properties and persistence in soils. Half-lives of the nitro-substituted neonicotinoids (including imidacloprid, thiamethoxam and clothianidin) range from about five to 25 weeks in soil, with some estimates ranging as high as two to three years. A general rule of thumb is that it takes approximately five half-lives to functionally eliminate a substance from the soil environment.
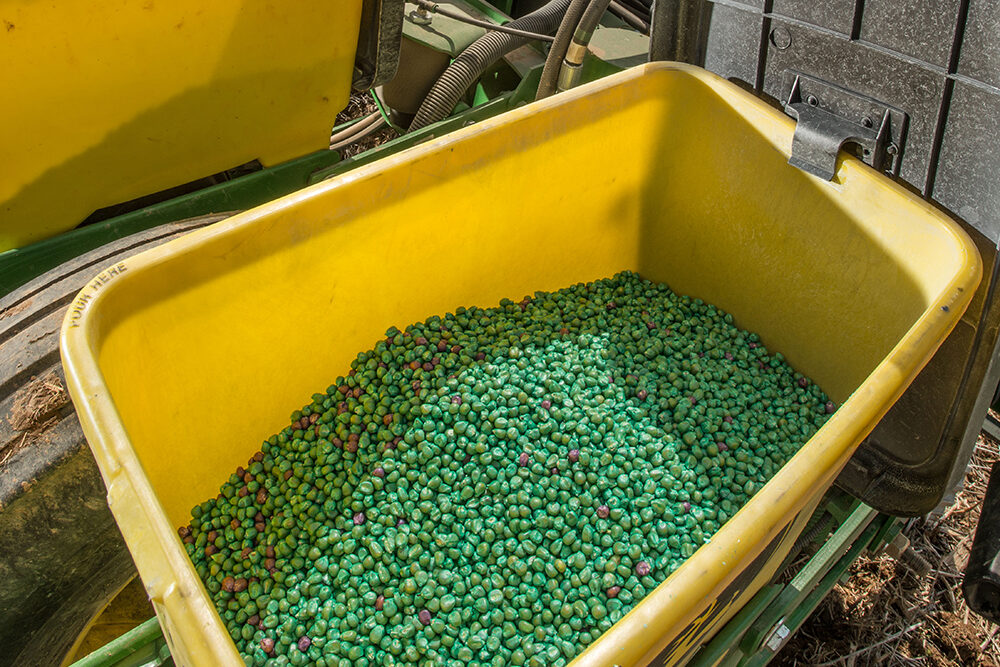
Neonicotinoid seed treatments can have complex effects on aboveground and belowground invertebrate communities. Only a small proportion (approximately 2–20%) of the active ingredient in neonicotinoid soil or seed treatments is taken up by plants; the bulk of the active ingredient remains in the soil and may pose risks to soil organisms. A meta-analysis of 20 field studies found that neonicotinoid seed treatments and pyrethroid insecticide applications had similar negative impacts on natural enemy abundance, reducing abundance of beneficial insects by an average of 16% compared with untreated fields. Natural enemies—including minute pirate bugs, lady beetles, lacewings, spiders, ground beetles and rove beetles— were found to be significantly less abundant in surface litter in clothianidin-treated corn plots than untreated plots. Loss of these natural enemies and other soil macrofauna could result in disruptions to the ecosystem services they provide, including controlling insect pests and consuming weed seeds.
Residual imidacloprid in the fallen leaves of treated trees inhibited earthworm and aquatic insect feeding and reduced leaf litter breakdown in two studies. These sublethal effects on decomposers could have broad implications for organic matter breakdown and nutrient cycling in terrestrial and aquatic ecosystems. Agricultural plant litter with imidacloprid residues may have similar feeding inhibition effects on decomposers.
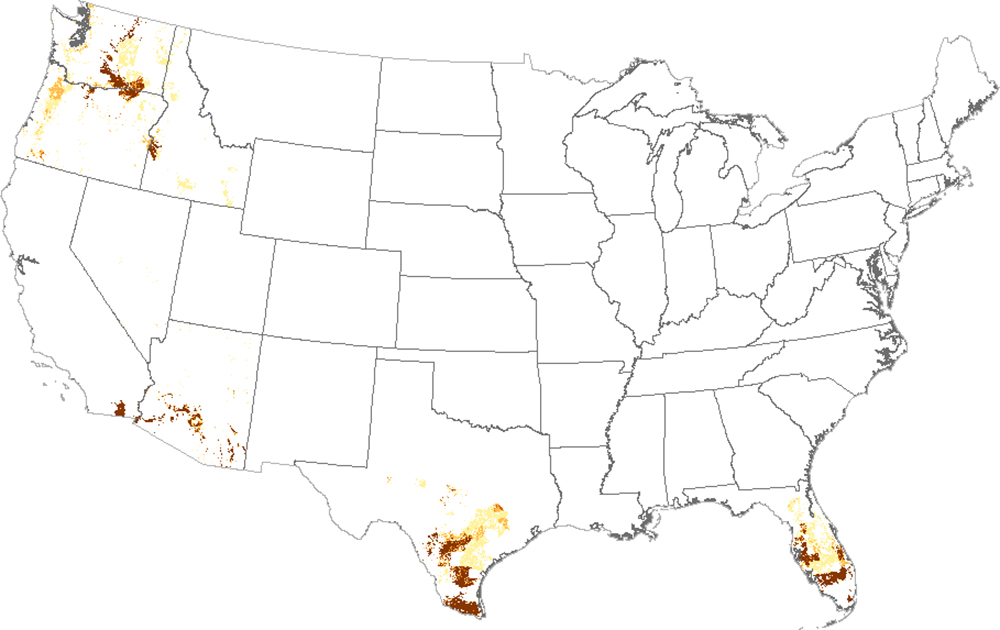
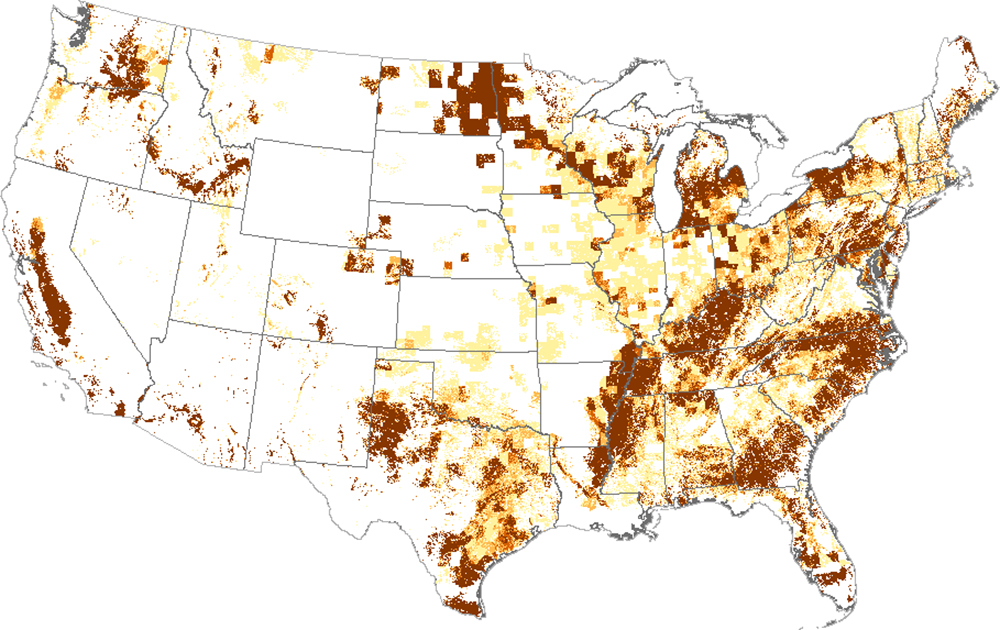
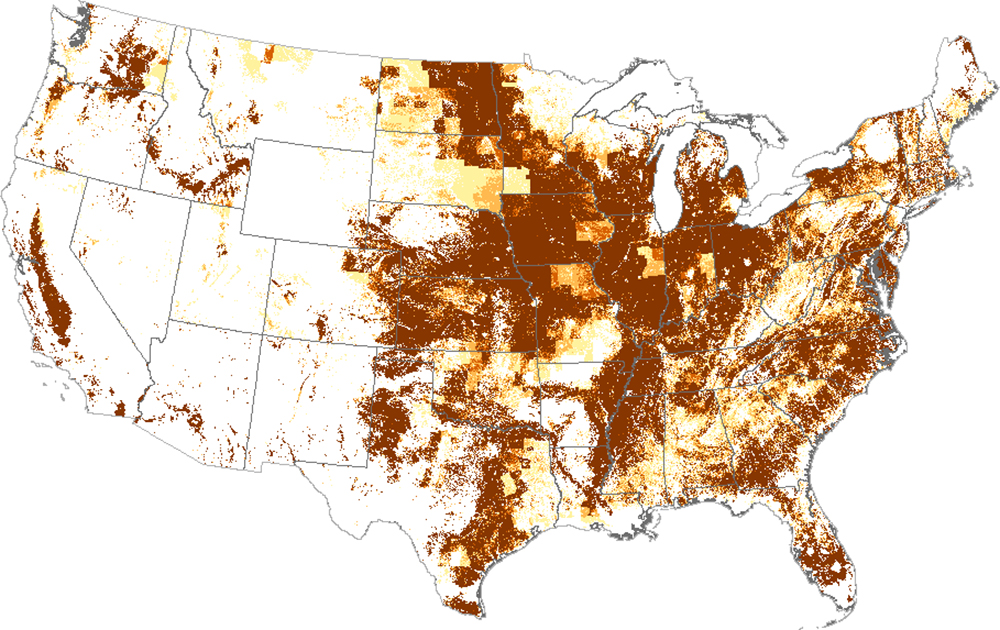
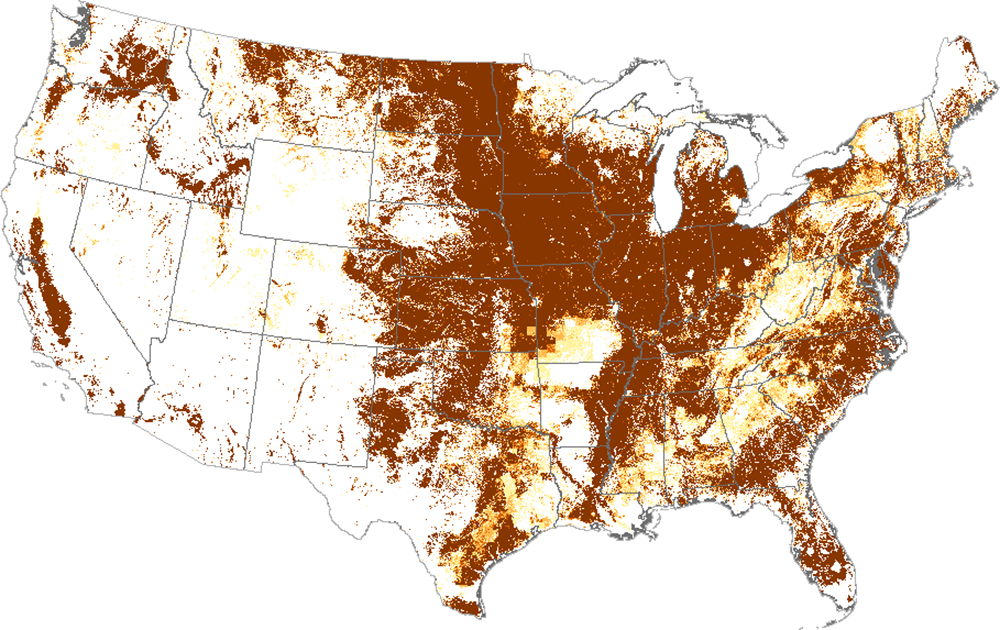
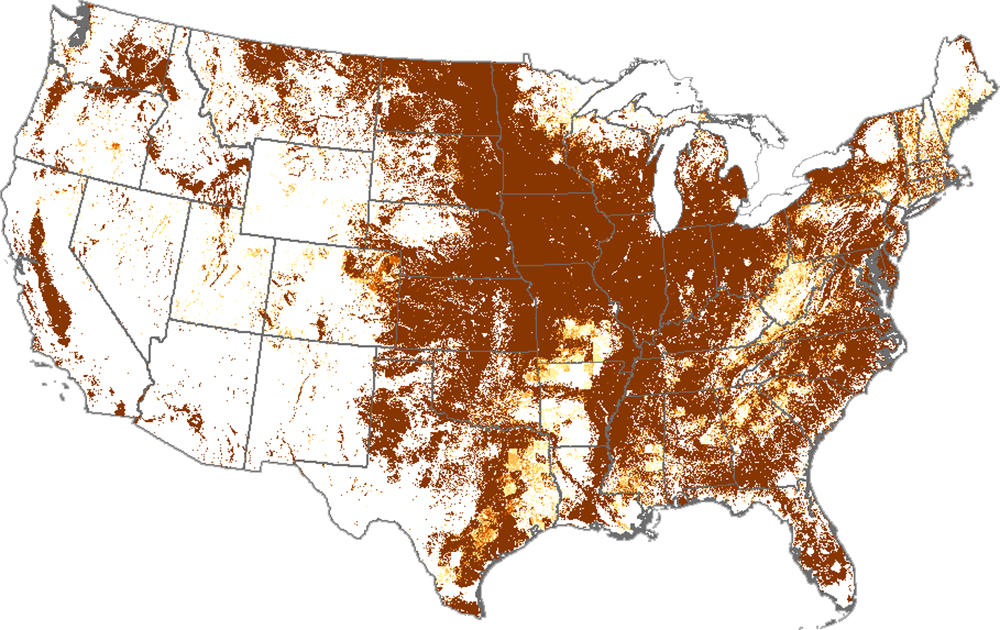
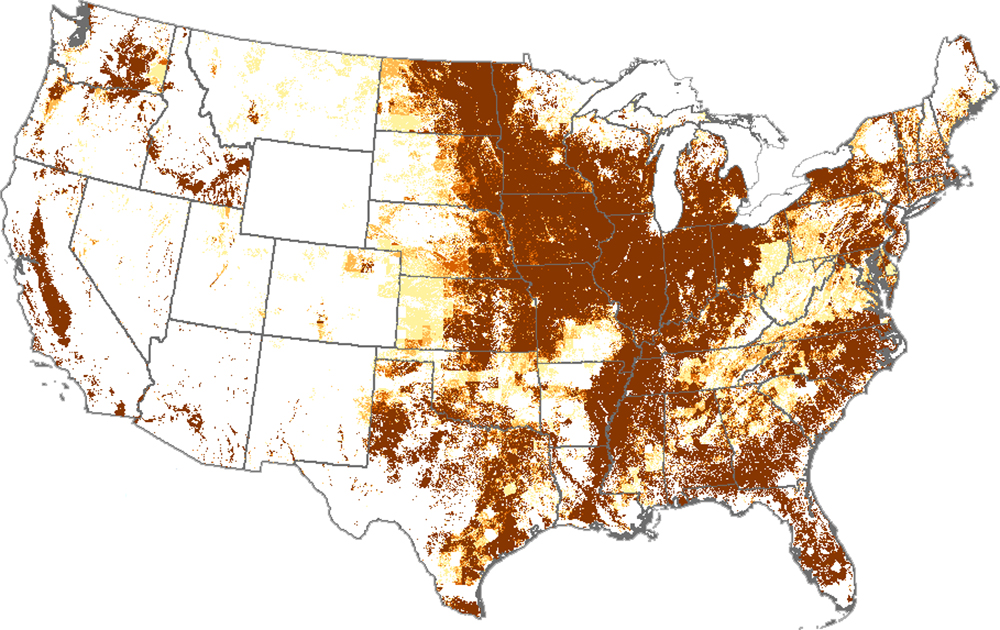
Figure 31—Estimated Annual Agricultural Use of Imidacloprid in the United States: 1994–2017 <0.01 lb/mi2 >0.04 lbs/mi2. Source
Fungicides
While insecticides are the pesticides likely to pose the greatest risk to soil-dwelling arthropods, fungicides may have larger impacts on soil microbial activity. Heavy metal fungicides (e.g., copper, zinc and sulfur) significantly reduced the biomass, activity and diversity of earthworms and soil microbial communities in orchard and vineyard soils. Soil and lab experiments have found that copper negatively affects survival and growth of earthworms, and that earthworms tend to avoid or move out of copper-contaminated soils, preferring soils with a lower copper concentration.
Fungicide applications that reduce earthworm abundance or activity may slow the breakdown of leaf litter that these soil fauna provide. Applications of the benzimidazole fungicides benomyl and thiophanate-methyl reduced surface earthworm populations and slowed leaf removal in apple orchards, with some residual effects on earthworm abundance lasting up to three years after benomyl applications.
Herbicides
Herbicides can have direct or indirect effects on soil invertebrates. Some herbicides, including paraquat and 2,4–D, can have direct sublethal or lethal impacts on a variety of beneficial insects, particularly their developing larvae. Glyphosate, the most widely used herbicide in the world, has been found to disrupt earthworm movement and reduce reproduction—although it is not clear whether these effects are due to direct toxicity or simply the loss of plant cover at the soil surface.
In some cases, exposure to herbicides may make invertebrates more susceptible to other pesticides they are exposed to in the soil environment. Triazine herbicides, such as atrazine and simazine, can synergize the toxicity of certain insecticides, particularly organophosphates. Atrazine has been found to increase the toxicity of the organophosphate insecticide chlorpyrifos to invertebrates by several fold. Glyphosate may synergize the effects of insecticide or fungicide seed dressings on earthworm activity.
Some herbicides, including atrazine, simazine, glyphosate and paraquat, can have a repellent effect on predatory ground beetles, which play important roles for pest and weed seed control in agricultural fields. In one study, beetles did not return to treated fields for a month after application of glyphosate or paraquat. This effect may be a response by these predatory insects to the loss of plant cover in herbicide-treated fields.
Indirect Effects
In general, in agricultural settings, herbicides are used to reduce aboveground plant diversity in favor of monoculture in order to reduce competition with the focal crop. Aboveground plant diversity drives the diversity and abundance of soil organisms—and vice versa—and so the loss of plants due to herbicide use is likely to negatively impact the diversity and abundance of soil-dwelling organisms that feed on pollen, nectar, seeds, leaves, roots and other plant tissues. Changing organic matter inputs may also affect populations of soil decomposers.
Soil Fumigants
Soil fumigants are pesticides that, when injected or incorporated into soil, form a gas that diffuses through the pockets of air in soils. Fumigants are typically used weeks to months before crop planting to control a broad range of soilborne pests, including insects, nematodes, bacteria, fungi and weeds. Many fumigants are toxic to a broad spectrum of soil life and will kill beneficial organisms along with soilborne pathogens. The use of fumigants can cause long-term disruption in the balance of soil communities—some organisms are more susceptible to fumigants than others, and some are better able to recolonize soils following fumigation. Interestingly, fumigants are generally far more harmful to mycorrhizal fungi than soil-applied fungicides, and in some cases have led to plant stunting due to elimination of beneficial mycorrhizal fungi in fumigated soils.
Knowledge Gaps
There are major knowledge gaps regarding pesticide impacts on most soil invertebrates and the ecosystem functions they provide and regulate. Many of the pesticides used have toxicity data for fewer than five soil invertebrate species. The current pesticide risk assessment framework in the United States fails to capture the diversity of responses and sensitivity to pesticides among soil invertebrates. The model organism for soil invertebrates that is typically used for lower-tier testing of pesticide toxicity, the red wiggler (Eisenia fetida), a habitat specialist earthworm, was chosen mainly for its amenability to laboratory testing rather than for its ecological relevance. Other soil invertebrates, such as springtails (Collembola), may be far more sensitive to pesticides than earthworms.
We also know little about community and population responses of soil invertebrates to the complex mixture of pesticides and their metabolites likely to be present in crop soils. Few studies have examined the interactive effects of different pesticide mixtures (e.g., whether mixtures are additive, synergistic or antagonistic in their toxicity to soil organisms).
Many pesticides can persist via a variety of binding mechanisms for months to years in the soil. While some residues are biologically inactive once adhered to soil particles, some remain bioavailable or can become bioavailable through interactions with soil microbes. There are few long-term studies examining the effects of chronic low-dose exposure to pesticide residues in the soil, including the metabolites produced as pesticides break down over time.
Recommendations
The first line of defense in managing pests is prevention. There are many cultural, biological, and mechanical methods that can break pest and disease cycles and keep pest populations at tolerable levels, reducing the need for pesticide applications. Use pesticides as a last resort if preventive strategies are not feasible, or if scouting and monitoring determine that pests have reached damaging levels despite preventive management. Do not apply pesticides before knowing if there is a problem. Neonicotinoid and other pesticidal seed treatments are often used without knowing whether the target pests are present or a problem in the fields where they are planted. Prophylactic use of these seed coatings across millions of acres of cropland is a waste of resources and poses a variety of environmental risks.
Scouting and monitoring can help determine what pests are present and whether populations are high enough to result in economic damage to crops. Once you know what’s in your fields, you should seek resources on pest life cycles and habitat requirements to figure out the best approaches for keeping their populations low over time. Think of it this way: if you have ants in the kitchen, you’d clean your sink and counters to remove the food sources that are attracting them. The same principle is true for removing food or shelter for insect pests and cleaning up infested plant materials in crop fields.
If you use pesticides, take steps to reduce the risk to soil life:
- Use the principles of integrated pest management to increase prevention-based strategies and reduce reliance on chemical management. Scout and monitor for insect pests and diseases and only apply pesticides if a pest has reached economically damaging levels.
- If using pesticides, target applications to the areas where pests are present. Reduced coverage methods like spot spraying and band or alternate-row applications can reduce pesticide use and cost while maintaining efficacy for a variety of crop pests.
- Do not use soil fumigants, which disrupt the balance and reduce the diversity of soil communities.
- Avoid tank mixing wherever possible, particularly mixes of insecticides, fungicides or herbicides that are known to jointly increase toxicity when applied together. The University of California IPM Bee Precaution Pesticide Ratings tool can help identify some of these synergistic mixtures.
- Choose less persistent and more selective options to reduce impacts on nontarget organisms.
- Minimize drift and off-site movement into natural areas near crop fields. These areas are refuges that allow soil organisms to recolonize crop fields after disturbances like tillage or pesticide applications. Consider use of electrostatic sprayers that improve deposition onto the target plant foliage and therefore reduce the amount of pesticide applied and the amount likely to reach soils.
- Follow all label instructions and apply pesticides at the lowest effective rate and frequency to minimize the amount reaching soils.
This material is based upon work that is supported by the National Institute of Food and Agriculture, U.S. Department of Agriculture through the Sustainable Agriculture Research and Education (SARE) program. Any opinions, findings, conclusions, or recommendations expressed in this publication are those of the author(s) and should not be construed to represent any official USDA or U.S. Government determination or policy.